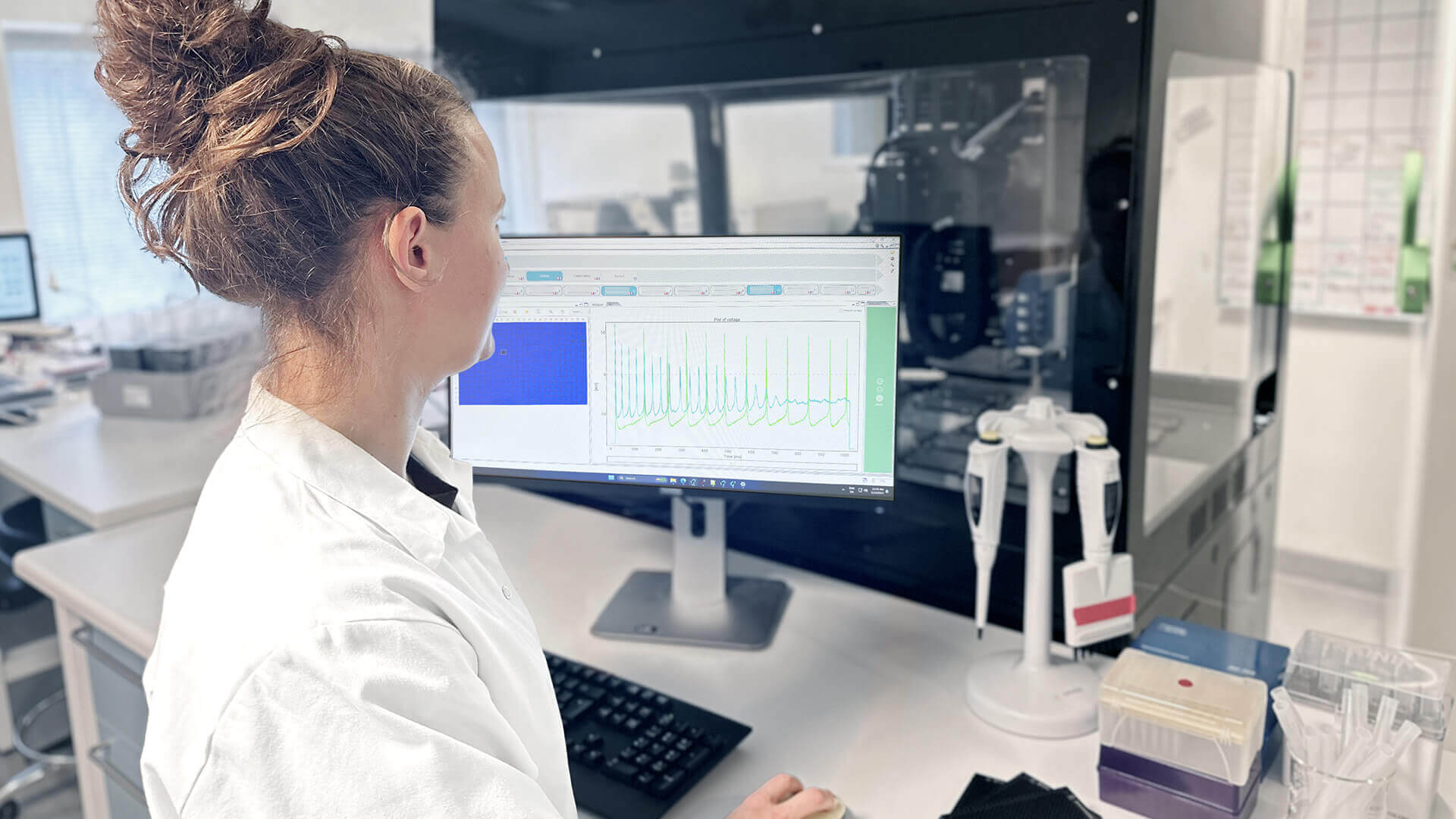
hiPSC-derived cell lines and automated patch clamp
Human induced Pluripotent Stem Cells (hiPSCs)
With the discovery of the Yamanaka factors in 2006 (REF: Takahashi and Yamanaka, 2006), mature somatic cells could be taken from adult tissue and reprogrammed to an embryonic-like, pluripotent state. The processes where pluripotent stem cells (PSCs) are differentiated and matured, allows fast-forwarding of cells into specialized cell lines, such as cardiomyocytes and neurons, providing model cells for in vitro research.
Originally hiPSC-derived cell lines were mainly considered for applications in regenerative medicine, but their extensive range of advantages have also made them interesting for the development of cell-based assays in the fields of drug-discovery, pharmaceutical research and personalized medicine.
Automated patch clamp measurements of hiPSC-derived cell lines
Ion channels represent attractive targets in hiPSC-derived cell lines, making electrophysiological studies important not only for characterization, but also for advances in biomedical research including drug discovery and development. The high-throughput (HT) nature of Automated Patch Clamp (APC) that enables recordings of large datasets within a few days or even hours, makes this method ideal for applications such as: quantification of inter-patient or inter-batch variability; identification of cell sub-populations within the same batch, based on their ion channel content; and HT drug screening.
Due to the improved quality and cell handling methods, ongoing development of APC features, and initiatives such as the Comprehensive in vitro Proarrhythmia Assay for cardiac ion channel safety pharmacology (Colatsky et al., 2016), the field have made significant progress in developing new APC applications for characterization and pharmacology measurements of hiPSC-derived cell lines.
Novel applications for APC studies of hiPSC-derived cell lines include:
Ion channel analysis (quality control):
A challenge of hiPSC-derived cells is that they typically display heterogeneous current expression. This might be due to different maturation states, different cell types, genetic, and/or biological variability. In APC, currents are recorded in parallel from many cells. This makes APC well suited to characterize cell populations and identify subpopulations.
A representative example is shown in Figure 1 in which a subpopulation of hiPSC-derived cortical neurons, recorded on Qube 384, is selected that expresses both Nav and a mixture of delayed rectifier and A-type Kv channels.
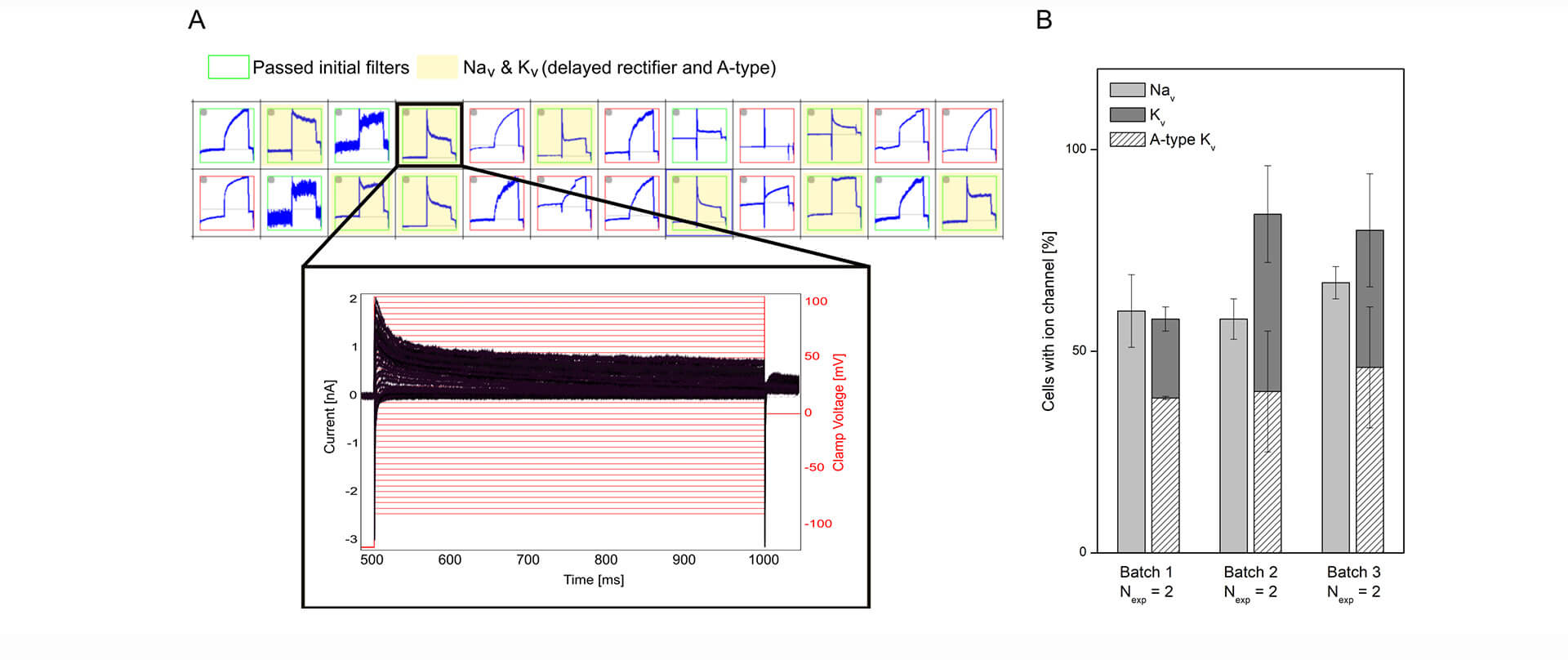
Figure 1: Characterizing mixed ion channel populations in hiPSC-derived cortical neurons using Qube 384. (A) Quality control filters allow selection of cells expressing the ion channels of interest. Top: Cut-out of the measurement plate view showing 24 of 384 experiments. Red and green borders correspond to experiments that failed and passed, respectively, the initial filter criteria. The experiments highlighted in yellow passed filter criteria for Nav and Kv currents. Bottom: Example of a population of current traces (black) elicited by + 5 mV voltage-steps (red) recorded from a neuron, expressing Nav channels and a mixture of delayed rectifier and A-type Kv channels. (B) The percentage (avg ± sem) of investigated cells containing Nav and Kv channels were displayed for three different differentiation batches of hiPSC cortical neurons (Nexp = 2 experiment days, with > 48 measurement sites per experiment).
Publications of ion channel analysis in hiPSC-derived cell lines include:
- Sophion Application Report:
hiPSC-derived cardiomyocytes – Nav1.5 compound screening on QPatch II
(hiPSC CMs from iBET) - Sophion Application Report:
hiPSC-derived cardiomyocytes: A robust and reproducible QPatch® II assay for Nav1.5 measurements
(iCell Cardiomyocytes2 from FUJIFILM Cellular Dynamics) - Sophion Poster:
Using automated patch clamp for high throughput characterization of sodium and potassium channels in human induced pluripotent stem cell-derived sensory neurons
(RealDRGTM hiPSC-derived sensory neurons from ANATOMIC)
Action potential measurements:
Measurements of cardiac and neuronal action potentials (AP) are of special interest for disease modelling, drug discovery and, specifically for hiPSC-CMs, cardiac safety. Measurements of APs in hiPSC-derived cell lines using APC are challenging, primarily due to immature phenotypes and heterogenous ion channel expression. However, optimized maturation, dissociation and purification protocols together with technological developments (such as adaptive current clamp) to compensate for missing currents, have made APC measurements of neuronal and cardiac APs possible, capturing disease phenotypes and compound effects.
hiPSC-CMs are notorious for being immature as compared to acutely isolated, primary cell lines. The immature phenotype includes the presence of the pacemaker current If (routinely expressed in immature CMs and lost in adult CMs) and reduced densities of hyperpolarizing current IK1. However, the maturation of hiPSC-CMs has improved, and the paradigm of impaired IK1 expression in hiPSC-CMs has been challenged (Horváth et al., 2018). In Figure 2 we display QPatch II measurements of “mature” IK1 currents in hiPSC-CMs.
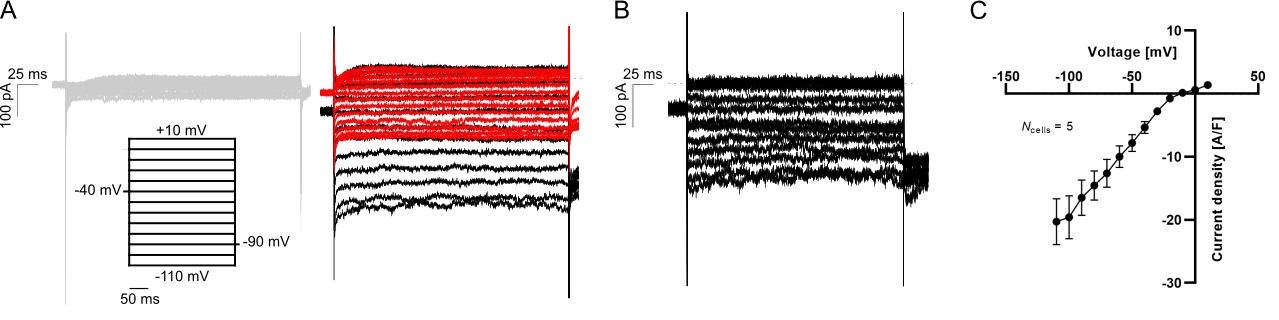
Figure 2: Measurements of IK1 current-voltage relationship in hiPSC-CMs using physiological solutions. (A) Representative K+ current traces in response to a voltage step protocol from –110 mV to +10 mV with 10 mV increment step size (black). The current was measured in 5 mM extracellular K+ (gray, left) and 75 mM extracellular K+ before (black) and after (red) addition of 1 mM BaCl2 (right). (B) The BaCl2 sensitive IK1 was calculated by subtracting the insensitive current (red trace in A) from the total IK (black trace in A). (C) The IK1 current-voltage relationship was plotted by extracting the average steady-state sensitive current (shown in B) and plotting as a function of step voltage.
An example of hiPSC-CM APs and compound effects, recorded in physiological solutions using QPatch II are shown in Figure 3.
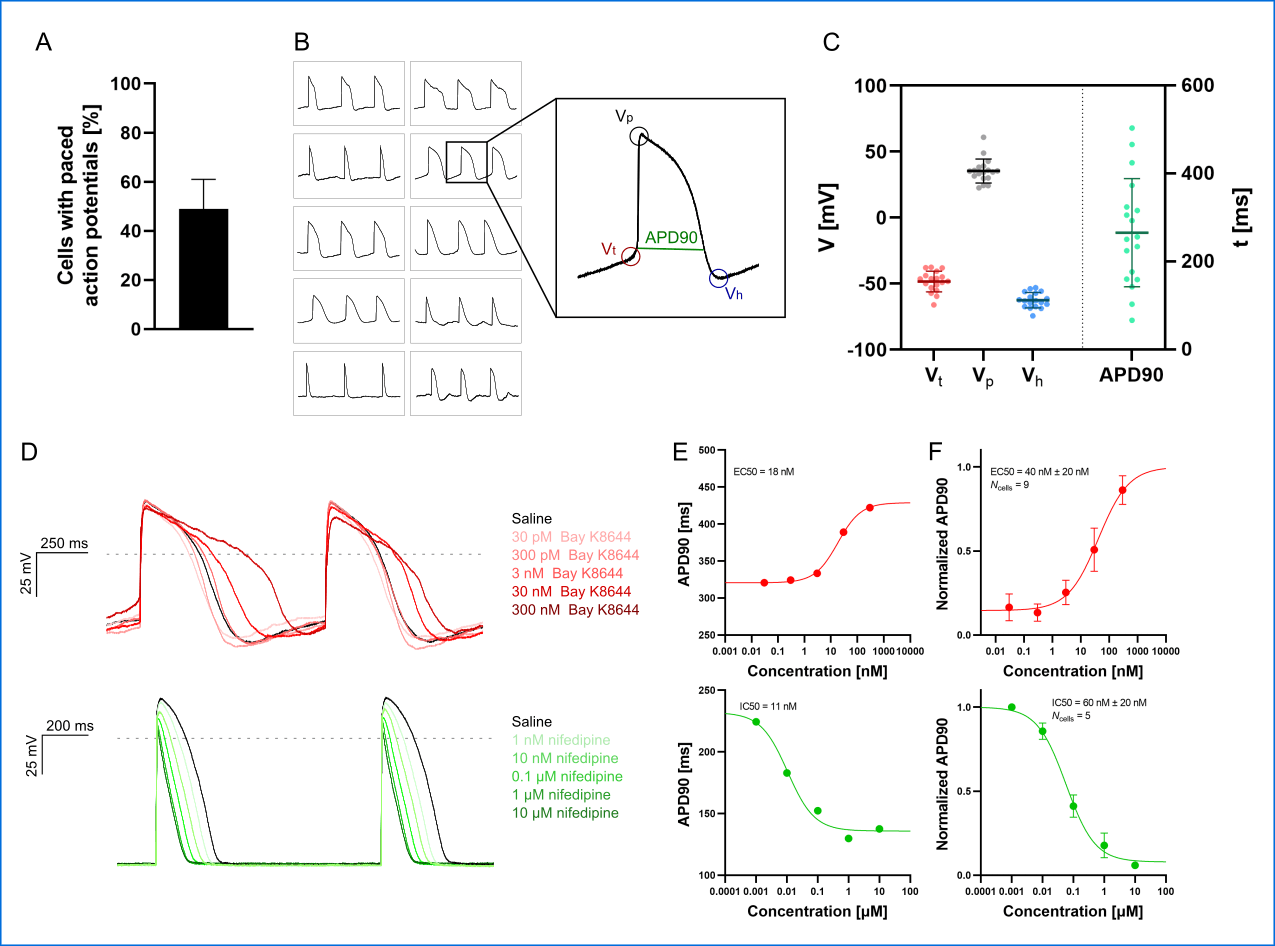
Figure 3: APC current-clamp measurements of paced action potentials and APD90 concentration-response plots. (A) The percentage of successful experiments displaying paced action potentials [Vp > 0 mV, resting membrane potential (RMP) < – 40 mV]. Data is avg ± sd of three measurement plates (each of 48 recording sites). Assay success rate was 10% – 30% out of 48 sites. (B) Paced action potentials from 10 individual hiPSC-CMs within a single measurement plate (left) and an expanded action potential displaying the extracted parameters: threshold potential (Vt ), peak potential (Vp), hyperpolarization potential (Vh), and action potential duration at 90% repolarization (APD90). (C) Plot of extracted parameters, Vt (red), Vp (gray), Vh (blue), and APD90 (green, ms) for 18 individual hiPSC-CMs with the avg ± sd (solid lines). (D) Paced action potentials for saline controls (black traces) and in response to increasing concentrations of Bay K8644 (top, traces in shades of red) and nifedipine (bottom, traces in shades of green). (E) Plots of APD90 versus compound concentration with Boltzmann fits including EC50 or IC50 calculated values, recorded in a single cell. (F) Cumulative concentration-response (average, APD90 normalized to pre compound concentration) relationships with Boltzmann fits including EC50 or IC50 calculated values. Data points are avg ± sem of Ncells.
Publications of AP measurements in hiPSC-derived cell lines include:
- Sophion Application Report:
hiPSC-derived cardiomyocyte recordings using physiological solutions on QPatch II
(hiPSC-CMs from the laboratory of Prof. Niels Voigt, UMG) - Sophion Poster:
Electrophysiological characterization of iPSC-derived excitatory neurons, including a comparison to genetically modified Frontotemporal Dementia neuron model, using automated patch clamp
(hiPSC-derived excitatory neurons from FUJIFILM Cellular Dynamics) - Sophion Webinar:
Automated Patch Clamp & iPSC: Manual and Automated Patch Clamp measurements of IK1 currents in hiPSC-derived cardiomyocytes
(hiPSC-CMs from the laboratory of Prof. Niels Voigt, UMG)
Disease modelling, drug-screening and personalized medicine:
hiPSC disease models have been developed for a range of diseases including neurodegenerative diseases, such as Alzheimer’s Disease and cardiovascular diseases such as long-QT syndrome. Although examples of APC applications within personalized biomedicine are still scarce, their need and value mean they are in active development with expectations high that we will see more in the near future.
An example of such an application is shown in Figure 4, in which an hiPSC motor neuron disease model, derived from a Spinal Muscular Atrophy patient (Faravelli et al., 2015) was characterized together with control cells from healthy subjects. The results showed the expected overactivity of Nav channels, which could be rescued by a Nav antagonistic compound addition (SMN-C3).
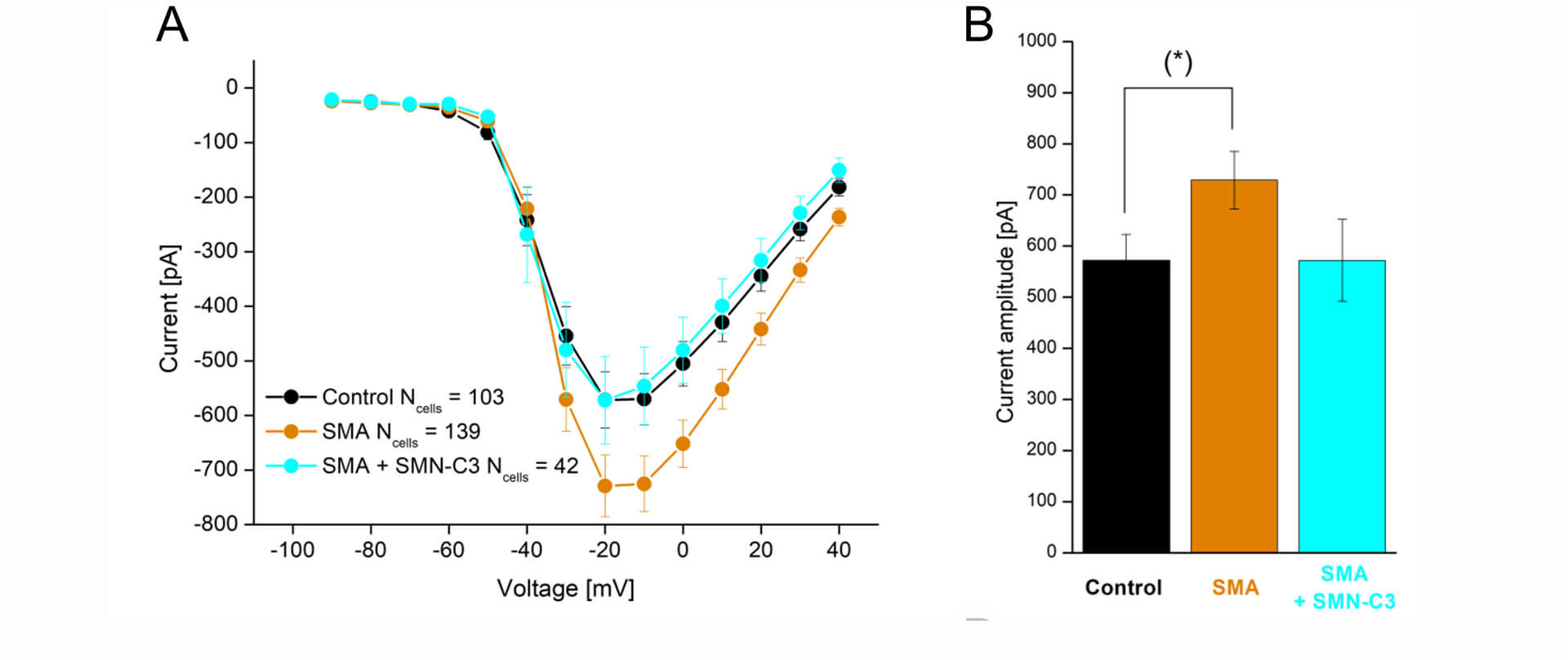
Figure 4: APC evaluation of compound effects on Nav channel properties in SMA hiPSC-neurons. Parallel recordings of control neurons (black), SMA neurons (orange), and SMA neurons treated with SMN-C3 during culturing (blue). (A) Average Nav current versus step voltage. (B) Quantification of the current amplitude at –20 mV. The current amplitude is significantly larger in SMA cells than in control cells [Student’s t-test, p < 0.05 (*), 95% confidence interval]. Error bars are SEM.
Publications of characterization of hiPSC-derived disease models include:
- Sophion Poster:
Electrophysiological characterization of iPSC-derived cortical neurons using automated patch clamp
(hiPSC cortical neurons from Boston Children’s Hospital) - Sophion Application Report:
Electrophysiological characterization of human iPSC-derived motor neurons using Qube 384 and QPatch
(hiPSC motor neurons from BrainXell)
Reprogramming, differentiation, and maturation of hiPSC derived cell lines
During the last decade, substantial effort has gone into developing protocols for generating specialized hiPSC-derived cells and tissues for applications within a range of areas, such as blood, smooth muscle, liver, heart, and brain (REF: Bellin et al., 2012; Shi et al., 2017; Rowe and Daley, 2019).
In general, the formation of different specialized hiPSC derived cells follow the same sequence of events: (1) the reprogramming (induction) of human unipotent somatic cells into hiPSCs by directed expression of certain transcriptional factors; and, (2) the differentiation and maturation of hiPSCs into specialized cells, typically by using signaling pathway agonists and antagonists in specific concentrations to induce stepwise progression through the embryonic development stages (Figure 1).
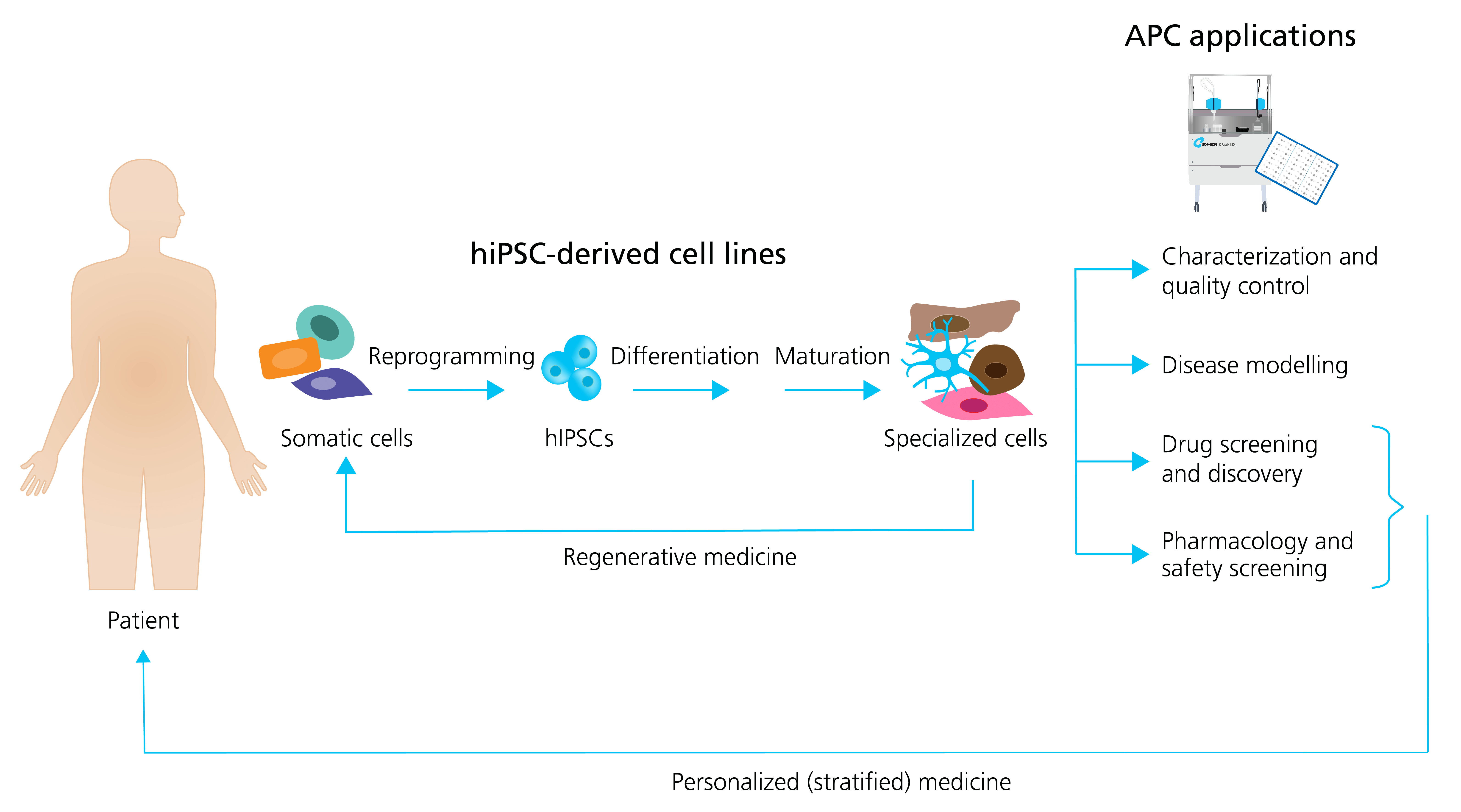
Figure 5: Generation of specialized hiPSC-derived cells and APC applications. Somatic patient cells can be reprogrammed into hiPSCs, followed by the differentiation and maturation into specialized cells. The specialized cells can be used directly in regenerative medicine, or they can be employed together with APC for a range of applications including: characterization and quality control; disease modeling; drug screening and discovery; and pharmacology and safety screening. Patient hiPSCs can be used as personalized drug screening and pharmacology models in trials. Adapted from Bellin et al. (2012), with permission from publisher Springer Nature.
Strategies to improve cell maturation during differentiation are many (see Figure 6) and include: the addition of specific chemical cues during differentiation; prolonged time in culture; electrical and mechanical stimulation (pacing); and finally modulating the chemical and spatial microenvironment of the differentiating cell culture, for example changing the adherence matrix, cell culture geometry, e.g., using 3D or preparing co-cultures with supportive cells.
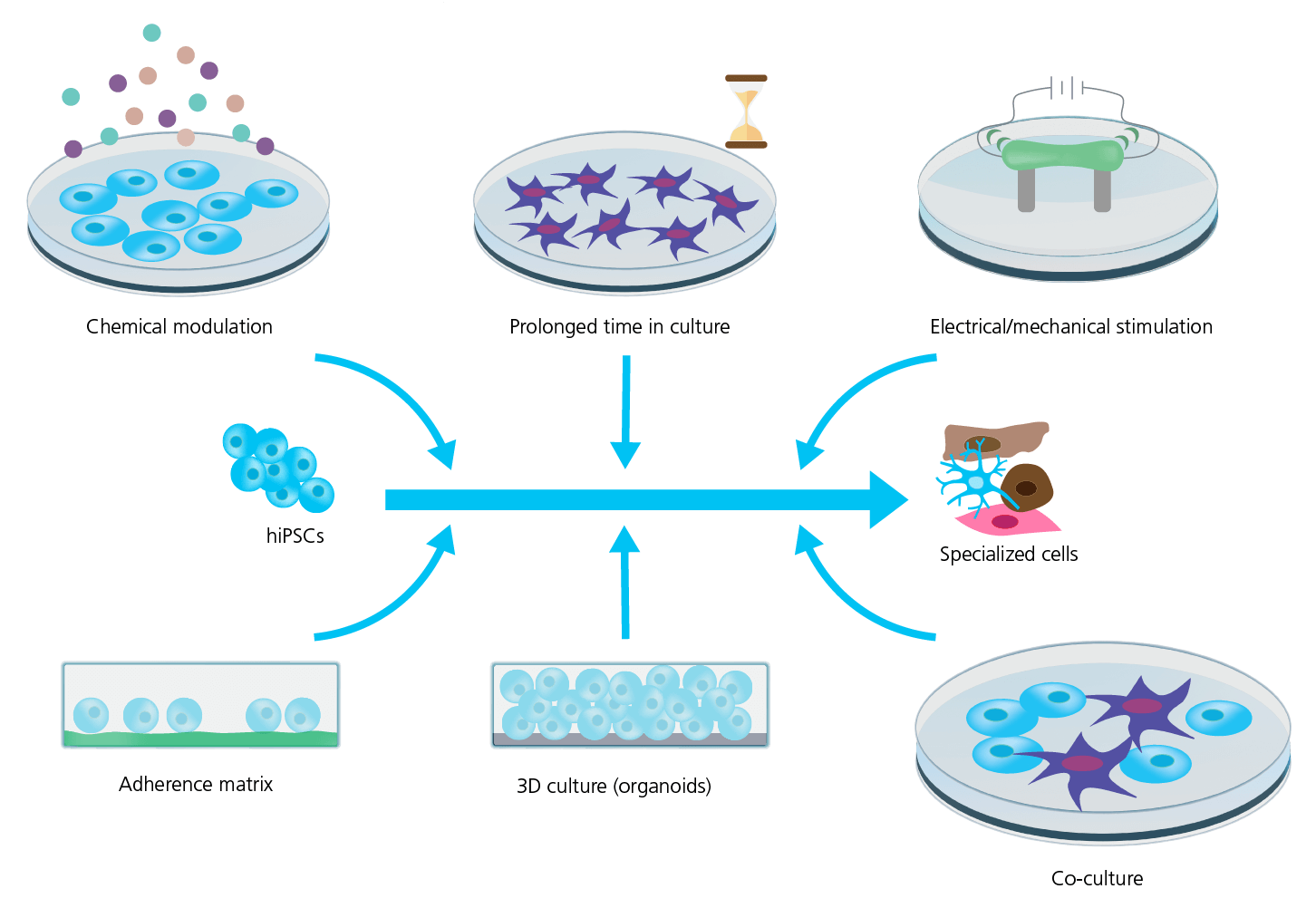
Figure 6: Strategies for improving hiPSC maturity. Several strategies are used to improve the maturity and phenotype specificity of hiPSC-derived cell lines. However, the mechanism and efficiency of these strategies are still being explored and requires rigorous characterization and quality control. Adapted from Ahmed et al. (2020), with permission under Creative Commons Attribution License (CC BY). 2021).
Dissociation and purification of hiPSC-derived cell lines for APC
For APC it is of outmost importance to work with a healthy, homogenous cell suspension that is free of debris. The preferred dissociation protocol will depend on the cell type, quality and culturing days and will likely evolve continuously (also, see Figure 7). Dissociating hiPSC-derived cell lines into single-cell suspensions is often challenging (especially CMs and neurons), due to the formation of tight cellular “tissue”-like structures and networks that must be disrupted. In general, the longer the cells have been cultured, the tighter the connections become, increasing the possibility of damage during cell harvest. Improvements involve: using gentle dissociating enzymes (e.g., TrypLETM, Gibco, United States; AccutaseTM, Stemcell Technologies, United States; or Papain, Sigma-Aldrich, United States); cold-harvest and/or incubation; addition of protective chemicals to ensure membrane integrity (e.g., ROCK inhibitor, blebbistatin) and prevent stickiness (DNase); replating for 24 h – 1 week; filtering to remove aggregates and possibly positive selection based on surface markers.
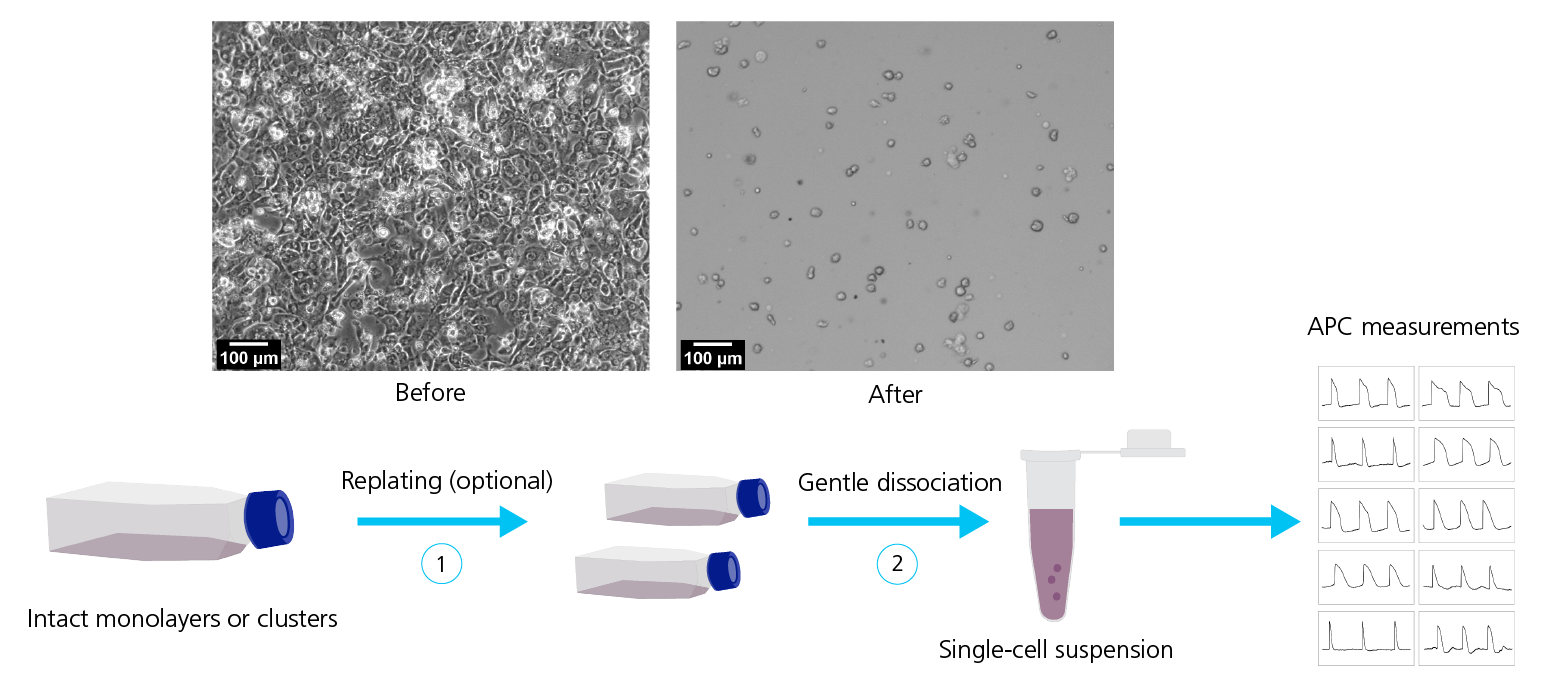
Figure 7: Dissociation of hiPSC-derived cell lines. Top row: Micrographs of hiPSC-CMs before (left) and after (right) dissociation. Bottom row: Dissociation of hiPSC-derived cell lines typically include a sequence of steps for example replating (1) followed by gentle dissociation methods (2) to obtain a homogeneous, healthy single-cell suspension suitable for APC measurements. Adapted from Rajamohan et al. (2016), with permission under Creative Commons Attributions License (CC BY).
Collaborators
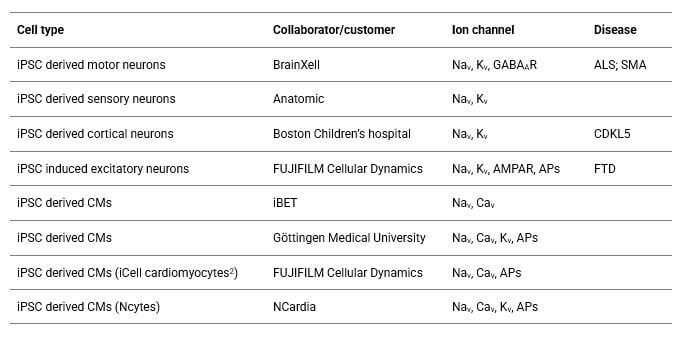
Click on the link below to view publications, videos, and application reports on iPSC cardiomyocytes and neurons.
Get in Touch
We strive to provide the best for our customers, and we are always ready to help. Please let us know if you have a question for us.